Key Takeaways
- There are major uncertainties about sentience, welfare ranges, and the taxa of interest that make it difficult to draw any firm conclusions.
- Nevertheless, it’s plausible that there are some differences in the probability of sentience and welfare ranges across insect life stages. However likely it is that adult insects are sentient, it's less likely that juveniles are sentient; however much welfare adult insects can realize, juveniles can probably realize less.
- These differences are probably best explained by the life requirements at those stages, which vary in species-specific ways.
- Insofar as we can compare the magnitudes of these differences, the best evidence currently available doesn’t support the conclusion that they’re large. For instance, we don't see a plausible argument for the view that adult insects matter 1,000x more than juveniles.
- We may be able to make some predictions about the probability of sentience based on two factors: first, degrees of independence at the immature life stages; second, the developmentally-relevant details of the rearing environments.
Introduction
This is the ninth and final post in the Moral Weight Project Sequence. The aim of the sequence is to provide an overview of the research that Rethink Priorities conducted between May 2021 and October 2022 on interspecific cause prioritization—i.e., making resource allocation decisions across species.
The goal of this post is to help animal welfare grantmakers assess the relative value of improvements to the lives of some commercially-important insects: the yellow mealworm (Tenebrio molitor), black soldier fly (Hermetia illucens), western honey bee (Apis mellifera), house cricket (Acheta domesticus), and silkworm (Bombyx mori). The vast majority of yellow mealworms, black soldier flies, and silkworms do not reach adulthood in the commercial systems of interest here (the exceptions are the members of the breeding population). By contrast, honey bees and house crickets do reach adulthood. This raises the question of how to value improvements to the lives of insects in their immature versus adult life stages. If adult insects are more likely to be sentient than immature insects, then, all else equal, it’s more important to prevent harm to adult insects than immature insects. Similarly, if it’s likely that adult insects can suffer more intensely than immature insects (which is relevant to their respective welfare ranges, which are one dimension of their respective capacities for welfare), then, all else equal, it’s more important to prevent harm to adult insects than immature insects. So, insofar as it’s possible to identify differences in either the probability of sentience or welfare ranges, that information is action-relevant when all else is equal. And insofar as we can compare the magnitudes of any such differences, that information may be action-relevant when all else isn’t equal.
This document unfolds as follows. In the next section, we provide some relevant biological information about the taxa of interest and introduce the reader to some important dimensions of the development and neurobiology of insects. After that, we explain how Rethink Priorities tried to estimate differences in the probability of sentience and welfare ranges for other taxa—which, among other things, involves surveying the literature for evidence regarding sentience- and welfare-range-relevant traits. We extend that approach here. Then, we summarize the literature we reviewed. Next, we argue for the key takeaways. Finally, we make some suggestions regarding future work in this area.
Biological Background Information
Insects develop in the larval (or nymphal) stage, undergoing a series of molts as structures develop; following one of two forms of metamorphosis, they emerge as adults with a fully grown exoskeleton. Holometabolous (complete metamorphosis) insects are those for whom the immature stage (larva) is a grub-like form with fewer structures and functions than the adult (e.g., caterpillars vs. butterflies). The pupal stage is the transition between those other two, involving the breakdown of larval structure before the insect emerges as a fully-developed adult (Hinton 1948). Hemimetabolous (incomplete metamorphosis) insects are those for whom the immature stage (nymph) is a smaller version of the adult, with many similar functions and structures that increase in size over the course of the life cycle (e.g., cockroaches). There is no pupal stage for hemimetabolous insects. The standard evolutionary theory about these two forms of metamorphosis is that incomplete metamorphosis came first, with complete metamorphosis arising 400 million years ago as a life history adaptation that enabled insect larvae and adults to live in different niches and use different resources (Rolff et al. 2019).
Of the species of interest, only house crickets are hemimetabolous. Cricket nymphs may go through as many as ten nymphal instars prior to adulthood, with each molt producing a nymph of progressively larger size (Patton 1978). In some of the holometabolous insects, the number of larval instars is variable and dietary or environmentally dependent (e.g., yellow mealworms; Morales-Ramos et al. 2010); in others, the instar number is typically considered to be fixed (e.g., black soldier flies; reviewed in Barrett et al. 2022a).
Figure 1. The general timeline, form, and stages in insects undergoing incomplete and complete metamorphosis in the cricket and soldier fly, respectively (figures from Fernandez-Cassi et al., 2019; Lievens et al., 2021). At comparable developmental points, the morphological and physiological differences between development types explain differences in needs and capacities.
Over the course of development, the specificity and complexity of many traits for species-specific functions will increase until adulthood, at which point the insect no longer grows due to its fixed exoskeleton (although plastic changes in internal anatomy are still possible; e.g., brain plasticity in adult honey bees, Cabirol et al. 2018, or neurogenesis in adult crickets, Cayre 1994). Given our interest in sentience and welfare ranges, of course, neurological development is of particular interest. Especially important will be understanding differences in the development of the mushroom bodies and their connections to other brain regions, as the mushroom bodies are of particular interest for insect sentience researchers (Barron & Klein 2016). The mushroom bodies are the ‘higher brain centers’ of insects and handle sensory integration, learning, and memory functions in adult and immature insects (Thum & Gerber 2019, Fahrbach 2006); they share a number of surprising similarities in structure, function, and development with the mammalian higher brain centers (e.g., Ganguly et al. 2020, Farris 2008) despite 600 million years of divergence.
The characteristics of neural development vary based on the type of metamorphosis an insect undergoes. In hemimetabolous insects, the nymphs have the same functionally discrete regions of brain tissue as their adult stages; these regions then grow incrementally over their development (Fahrbach 2006). For example, nymph and adult termites all have similarly structured, functionally-discrete regions for visual input (optic lobes), olfactory input (antennal lobes), and the same mushroom bodies (O’Donnell et al. 2021). By contrast, in holometabolous insects, these same brain regions have not obtained their final structural form (as in adulthood), although they can still generally be differentiated. In addition, the cell populations/connections may change more substantially in many holometabolous insects, both over the course of immature development (Fahrbach 2006, Farris et al. 1999) and especially during pupation (Zhao et al. 2008). In particular, holometabolous insects undergo a taxon-specific process of mushroom body reorganization during pupation that may be mild (some axonal degradation in particular cells) or involve the entire retraction and regrowth of whole mushroom body lobes (Fahrbach 2006). This extreme reorganization of the mushroom bodies appears to be exclusive to the holometabolous insects; in crickets and cockroaches, no reorganization of the mushroom bodies occurs at the end of nymphal life (Malaterre et al. 2002, Farris & Strausfeld 2001).
The concept of neural reorganization during metamorphosis, as well as the simplified structural appearance of larval brains, may lead some to think that there are significant enough differences between larval and adult holometabolous insect neuroanatomy to deny that insects are sentient in the larval life stages. However, many sentience-relevant features exist within seemingly minimal larval brains. For example, the expression of genes encoding some degree of response to painful stimuli have been demonstrated in the orders Orthoptera (crickets), Diptera (flies), Coleoptera (beetles, like the yellow mealworm), Lepidoptera (moths, like the silkworm), and Hymenoptera (bees), in both immature and adult stages (Kohno et al., 2010; Lambert et al., 2021; Milinkeviciute et al., 2012). In addition, sensory structures enable neurotransmitter-induced responses to noxious abiotic stimuli, such as heat exposure and mechanical stimulation; these abilities have been demonstrated in larval stages of Drosophila melanogaster (flies) and Manduca sexta (moths) (Guo et al., 2014; Im & Galko, 2012; Milinkeviciute et al., 2012; Neely et al., 2010; Verlinden, 2018; Walters et al., 2001; Zhong et al., 2010). Information (including about noxious stimuli) learned during larval life stages can even inform behaviors in adulthood in many holometabolous insects, including flies, weevils (in the same family as beetles), wasps and ants (in the same family as bees), and moths (Blackiston et al. 2008, Ray 1999, Tully et al. 1994, Reitdorf et al. 2002, Gandolfi et al. 2003, Isingrini et al. 1985, but see Barron & Corbet 1999), suggesting that reorganization of mushroom body synaptic connections is not so substantial in all taxa as to preclude the persistence of larval memories beyond metamorphosis.
Immature insect brains are smaller than their adult counterparts, in terms of total brain volumes and cell numbers (of which neurons are one kind). Presumably, the cognitive processes that result in sentience are products of the cells and synaptic connections that form the brain tissue. So, we might be tempted to conclude that having fewer of these neural units would mean these immature life stages are less likely to be sentient than their respective adults. However, as we've argued elsewhere, using total brain size or cell numbers are unlikely to result in accurate assessments of the probability of sentience across life stages. A large body of evidence suggests total brain volume can be a poor indicator of cognitive/behavioral repertoire (reviewed in Chittka & Niven 2009), including within a single species (Muratore et al. 2022), and thus is likely a poor indicator of probability of sentience. Increases in brain size may be driven, for example, by larger cell sizes for biophysical reasons – these larger cells have no obvious role in the production of sentience compared to smaller cells. Larger cell numbers might seem more relevant, then – but neural network analyses demonstrate that very limited cell numbers are needed for many of insects’ complex cognitive processes (e.g., Howard et al. 2022 found that only 5 cells would be required to correctly categorize even/odd numbers up to 40). Thus, the relatively-fewer cells necessary for these complex tasks may be drowned out in total brain cell estimates by the many more repetitive cells that are used, for example, to process the color, motion, and brightness of visual information from each eye’s photoreceptors in the optic lobes (Chittka & Niven 2009).
On a related note, we might suppose that the differences in brain volume or cell number across life stages are going to be largest in regions of the brain that are unlikely to be responsible for sentience, but which are instead related to other changes in environment/behavior. Consider the findings of Barrett et al. (2022b), who provide an estimate of the number of cells in black soldier fly larval and adult brains. Total brain cell numbers increased 16.2-fold from the last larval stage to the adult stage (approximately 20,000 to 330,000 cells). However, the vast majority of this increase in cell number occurs in the optic lobes - structures that are unlikely to play a role in producing valenced mental states, but which accounted for 87% of total adult brain cells and 74% of total brain mass.
Optic lobe increases occur in adult black soldier flies to support their behavioral transition from flightless to flying insects. By contrast, we have already demonstrated that the brain region most likely to be associated with sentience (the mushroom bodies) supports behaviors during both immature and adult life stages. Thus, if it’s the case that black soldier fly adults have the neural structures required to produce valenced mental states (which, of course, they may not), the small difference in non-optic-lobe brain cell numbers between adults and larvae provides little reason to think these structures would be absent in larvae.
Unfortunately, we don’t have similar comparative data for other species and we see no plausible way to estimate larval vs. adult brain cell numbers from other known values, such as body mass. It’s possible, though, that there are larger differences in brain cell numbers between the final immature and adult stages of other taxa. However, there are two important points to note here. First, there are general reasons to be skeptical of this possibility in some cases. For instance, non-flying holometabolous insects may see less substantial optic lobe growth during pupation (e.g., adult ants have lower cell numbers and densities for their body size than flying bees/wasps, likely due to optic lobe differences; Godfrey et al. 2021), which may reduce changes in total cell numbers during the transition to adulthood. Many hemimetabolous insect brains often grow more incrementally, and from a larger starting number of cells following embryogenesis (e.g., A. domesticus mushroom body neuroblasts, Urbach and Technau 2003). In both cases, then, we may find even smaller differences than in black soldier flies.
Second, suppose that future research were to reveal that honey bees have larger differences in brain cell numbers between their final immature and adult stages. After discounting the optic lobes (which are a large proportion of the cells in the adult stage for black soldier flies, but likely to be a fairly small proportion of the 20K cells in the final immature stage, given the low level of visual information processed by larvae), it’s reasonable to assume that adults have roughly 2 - 4 times as many potentially-sentience-relevant brain cells as their larvae. Given that insect brains in even some of the largest and most cell-dense Hymenoptera (bees, wasps, ants) approach an upper limit of two million neurons, when including their optic lobes (Godfrey et al. 2021), what is an absolute upper bound estimate of how much larger the differences could be? At the limit, two orders of magnitude? Then, we would get a 200-400x difference in potentially-sentience-relevant brain cell numbers. As we’ve argued, there are good reasons to be careful about the inferences we draw from differences in total brain cell numbers; so, a 400x difference does not imply the same difference in the probability of sentience. Moreover, it’s even less clear what it implies about differences in the probability of sentience across taxa. That is, even if adult honey bees were 400x more likely to be sentient than final immature stage honey bees (which we very much doubt), it wouldn’t follow that adult honey bees are 400x more likely to be sentient than the adult members of another taxon with 1/400 as many brain cells. After all, consider that the brains of some miniature parasitoid wasps have roughly 4600 cells (Polilov 2012) and, as far as we know, have comparable behaviors to larger-bodied Pepsis wasps, which have 1.8 million brain cells (Godfrey et al. 2021) –a ~400x difference in brain cell count. So, while information about such differences would be valuable to have, it’s important not to overstate its potential significance for either intra- or interspecies cause prioritization.
With this background information on developmental neuroanatomy in place, we turn to the traits that Rethink Priorities has considered elsewhere to assess relative differences in both the probability of sentience and welfare ranges.
The Trait Survey
In 2019, Rethink Priorities published its Invertebrate Sentience Table, which canvassed a wide array of affective, behavioral, cognitive, and physiological traits that might be taken to be relevant to the probability of sentience—which, for present purposes, we’ll understand as the probability that individuals can realize any welfare at all. Rethink Priorities has also published a Welfare Range Table to investigate potential differences in capacity for welfare. An organism’s capacity for welfare is its welfare range multiplied by its lifespan. Given hedonism, an organism’s welfare range is determined by the strengths of the valences of the positive and negative states that an organism can realize. Or, more colloquially, part of an animal’s welfare range is the difference between how well or badly things can go for that animal at any given time. (For more on this notion, see the Introduction to the Moral Weight Project.)
The traits that bear on an organism’s probability of sentience are those that indicate whether the organism can realize any welfare at all. If an organism lacks nociceptors, for instance, that provides good reason to lower our probability of sentience estimate for that organism. However, the presence of nociceptors doesn’t have any obvious implications for welfare ranges, as their presence alone doesn’t suggest that animals can have states with stronger positive or negative valences.
Which traits do bear on that question? While there are significant uncertainties here, a hypothesis worth taking seriously is that, all else equal, organisms with greater representational sophistication and processing power may be able to realize a wider range of welfare states than organisms with less. There are different routes to this hypothesis, including the possibility organisms may acquire richer representational abilities partly to rank the options available to them and the idea that processing power may enable the production of more intensely valenced experiences (Schukraft 2020). For present purposes, though, we’ll make the crude assumption that welfare ranges increase with behavioral complexity, as that’s all we’re able to assess in the species and life stages in question.
Rethink Priorities’s Invertebrate Sentience Table and Welfare Range Table identify nearly 150 empirical-detectable traits that are potentially relevant to these properties. Unsurprisingly, our literature review revealed that many of the traits in both tables haven’t been studied in the taxa of interest. All told, we were only able to find direct evidence for 19 of the traits for any taxon in any life stage, where that evidence was either positive or negative and was assessed via Google Scholar queries from November 2021 to April 2022 (Table 1). By “direct evidence,” we mean research done on the species in question, rather than research done on related species from which it’s possible to infer, with varying degrees of confidence, certain conclusions about the taxon of interest. For the sake of making the project manageable—and since we don’t think that this additional research would change the basic points we make here—we’ve opted to ignore such indirect evidence.
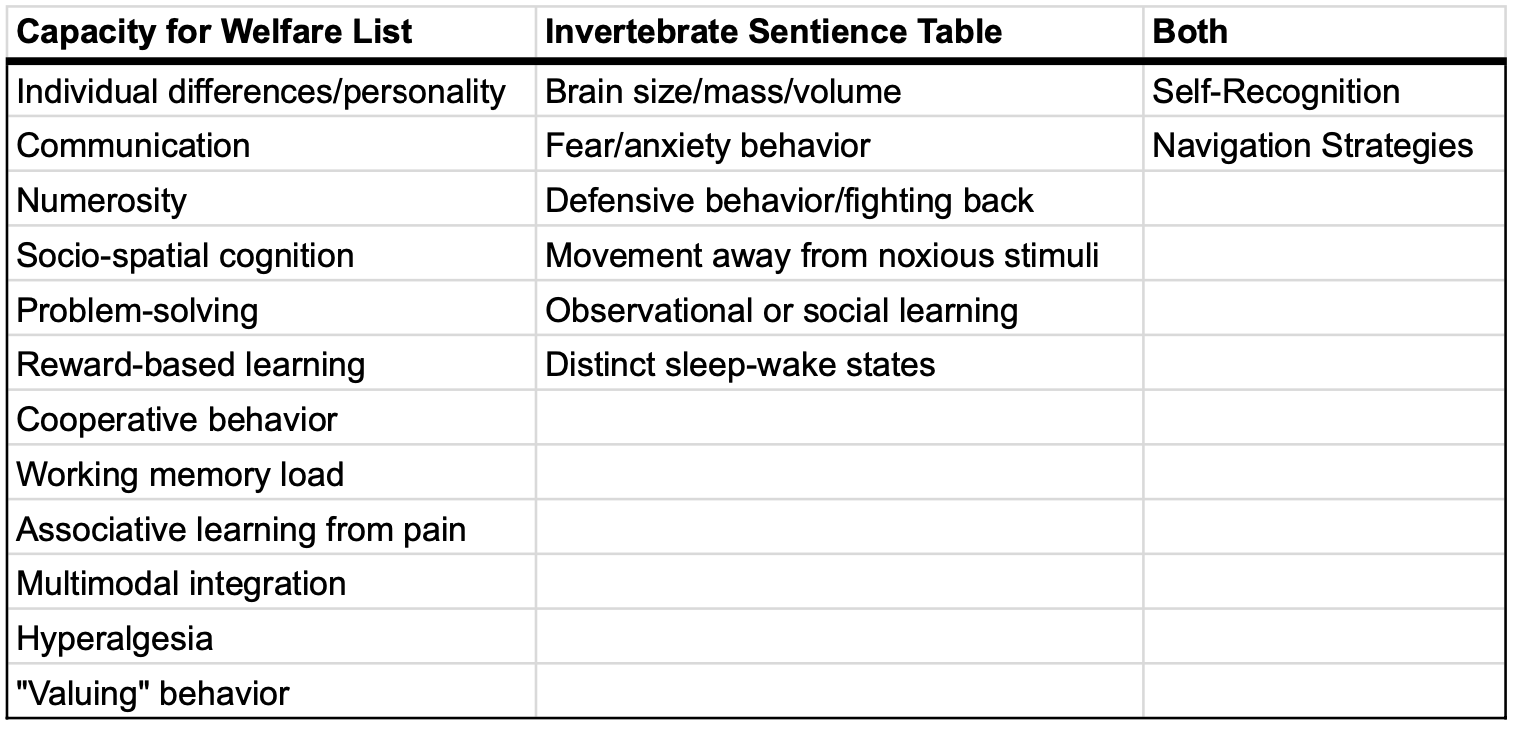
Table 1: The list of traits for which we found published evidence assessed via Google Scholar queries from November 2021 to April 2022
Again, we based our assessments on all the traits for which we could find some direct evidence. It’s important to recognize that this biases our results against larvae/nymphs. This is because insofar as the relevant traits have been studied, they often haven’t been investigated across the lifespan: data on adults is much more common than data on immature life stages. In many cases, we suspect that the best explanation for this disparity—just like the best explanation for many of the gaps in the adult data—is that no one has yet bothered to look. We considered alternate approaches for handling the bias against immature life stages, but ultimately saw no plausible basis for excluding any of the direct evidence we found; so, we’ve opted to proceed with all the evidence and qualify our conclusions accordingly.
We should also note that we decided to treat information about total brain cell counts differently than information about the other traits. Because it’s the only trait that we can compare quantitatively between life stages, it threatens to dominate the other results, even though it isn’t at all clear whether it should. (See RP’s report on this topic.) To avoid this issue, we opted to provide information about brain cell counts as background rather than including it in the table that we use to summarize our results.
Summary of the Literature on the Traits
An annotated review of the literature on the physiological ontogeny and life cycle for each species of interest is available here and the data sources we used for scoring the traits we present in this summary can be found here. Table 2, below, summarizes the results. Given how limited the evidence is, we opted to present a single table that includes all the traits relevant both to welfare ranges and to probability of sentience. Moreover, given our uncertainty about how to weight the traits we considered, we adopted a simple scoring rubric that assigns them equal weight, making it possible to consider a very crude quantitative estimate of the differences between life stages and taxa. We converted the findings in the literature into a matrix of relevant trait presence, absence, and knowledge gaps; then, we scored the traits as follows: 1 for present, -1 for absent, and 0 for those where a particular taxon-trait-life-stage triplet hasn’t been examined. We summed the values for each taxon / life stage combination.
Table 2: Scoring of trait evidence in the literature for species of interest. Scores of 1 represent trait presence; -1, trait absence; and 0, trait unknown. The green and blue columns include the traits assumed to be relevant to the probability of sentience; the yellow and green columns include the traits assumed to be relevant to welfare ranges. Supporting information for this table can be found here.
Crucially, we are not presenting these numbers as relative probability-of-sentience estimates; we aren’t suggesting that adult crickets are 250% more likely to be sentient than adult silk moths. First, the links between these traits and the target features (sentience and welfare ranges) are too tenuous. Second, there are too many holes in the data. Nevertheless, we can use this table to get some perspective on the findings of the literature review, as we’ll illustrate below.
Key Conclusions
We take the above to support four conclusions.
There is considerable uncertainty
This project is plagued by significant empirical, philosophical, and methodological uncertainties. It is essential to keep this in mind when assessing the evidence regarding the relative probability of sentience and potential differences in welfare ranges.
On the empirical front, while the morphology and physiology of the insects of interest have been researched, the degree and depth with which they’ve been documented or tied to behaviors at each life stage is highly variable. For instance, even though defensive behavior and directional memory are critical to locating resources and responding to stimuli, such as food, they essentially haven’t been examined in most insects’ immature stages.
Admittedly, there are challenges unique to the subject matter of sentience/welfare in immature insects, particularly in the holometabolous insects. Consider, for example, that expressions of defensive behaviors may be clearer in adult organisms with more complex body morphology or greater mobility, which allow for a greater range of responses to different stimuli than in the larval life stage. This dramatic variation in morphology can explain intraspecific variation in defense responses across life stages, making it even more challenging to correctly interpret larval behaviors. This can even be the case in hemimetabolous insects, such as crickets, which may gain the ability to fly only following their final molt, and thus adults have a different behavioral repertoire available when responding defensively to stimuli. Still, we should note that tests across life stages in field crickets (Gryllus integer) demonstrate that the same ‘immobility/freezing’ defense behaviors can occur at all life stages – and that small nymphs actually engage in those behaviors for longer periods than adults (Hedrick & Kortet 2012) – providing some reason to think that not all the missing data can be chalked up to the difficulty of collecting it. Thus we think it’s likely that many of the gaps in the literature are explained by the absence of the right kinds of academic incentives.
On the philosophical front, there is deep disagreement among experts about the merits of radically different theories of consciousness, some of which clearly imply that insects are conscious and some which clearly imply that they are not conscious. Similarly, there are ongoing debates about the best theory of welfare and its implications for differences in capacity for welfare.
On the methodological front, we had to limit our investigation to just a handful of traits based on the limits of the literature. As the authors of the Invertebrate Sentience Report make clear, there are many reasons to be cautious about their conclusions, which are based on much richer datasets. Those qualifications apply all the more to this report.
As a result, all our conclusions are tentative and could be significantly revised with further theoretical and empirical research.
There are probably some differences
Nevertheless, there do appear to be some probability-of-sentience- and welfare-range-relevant differences across life stages. Although we suspect that many unknowns about immature insects are due to no one having bothered to do the research, it’s unlikely that that’s the explanation for every gap in the table: presumably, some are based on reasonable judgments by experts that investigation won’t yield a positive result. So, insofar as differences with respect to the selected traits provide some evidence of differences with respect to the probability of sentience and welfare ranges, it is reasonable to infer that there are some differences along these axes.
This fits with more general considerations about differences in the species of interest across life stages. The mealworm, for example, spends the majority of its life cycle in the larval stage. In this stage, it senses and responds to stimuli, such as light perception, but primarily stays in one place (buried in its feeding substrate). It can assess and discriminate between resources in its localized environment (e.g., food, Murray 1960) but usually will not need to seek them out at great distance and thus avoids many types of complex environmental stimuli. After metamorphosis, it becomes a beetle. This new life stage requires locating a mate and resources, tasks that require more complex perceptual and sensory abilities in order to navigate the more complex environment. These basic facts suggest that, throughout development, either (a) some capabilities that may be sentience- and/or welfare-relevant only come online at particular stages of development or (b) that those capabilities are enhanced from life stage to life stage.
The best evidence doesn’t support the conclusion that the differences are large
That being said, insofar as we can compare the magnitudes of these differences, the best evidence currently available doesn’t support the conclusion that they’re large.
First, when we consider the scores in Table 2, all the differences are within an order of magnitude. Granted, this is an artifact of the scoring system. But the choice of scoring system isn’t arbitrary. If we knew how to weight the various traits—e.g., that associative learning from pain is 100x more important than multimodal integration—then we could have used a scoring system that would have generated larger differences. Since we don’t know how to weight them (and no one else does either), it makes sense to treat the current scoring as our best estimate of the sizes of the differences that can be justified based on the information now available. Of course, as we ourselves argued, there is considerable uncertainty here. So, our estimate is not an attempt to fix an upper bound. Still, even acknowledging the data we’ve surveyed could suggest that immature insects of some species may be less likely to be sentient than their adult forms (particularly in crickets and honey bees), the significant problem of empirical uncertainty due to large gaps in the literature suggests that there’s no plausible empirical basis for radically discounting immature insects relative to adults (insisting, e.g., that one larva is worth 0.00001 adult) at this time.
Second, this conclusion fits with the neurological and behavioral evidence discussed in section 2. The final immature stage of the species of interest will have some mushroom body development - enough to support learning and memories that can even persist across metamorphosis, in some tested species. Insofar as we understand the mushroom bodies to be broadly relevant to sentience as the ‘higher order center’ of cognition, there is no reason to assume (given current data) that particular differences in the mushroom body cell populations or numbers across these life stages would change the probability of sentience or welfare ranges of immature insects relative to their adult forms. Evidence on changes in total cell number across metamorphosis in black soldier flies does not support the idea that the vast majority of the increase in cell numbers is occurring in potentially-sentience-relevant regions (although, again, only a few cells are often needed to support many cognitively complex functions, and thus total brain cell numbers are a poor proxy for cognitive capabilities, broadly), though of course more data from other species would be required to draw more certain conclusions here. Still, the general neural structures present in many final stage immature insects and their adults are both capable of supporting some similar, potentially-sentient-relevant behaviors, and we have no evidence at this time to support the idea that any particularly important, sentience-responsible neural structures are absent in immature insects.
There are some factors that may help us make predictions about differences
Although there is considerable uncertainty about both the probability of sentience and relative welfare ranges, there may be some ways to make better relative judgments. For instance, immature insects vary in the degree to which they interact independently with their environment. In some immature insects, their food, shelter, and a degree of security from predators/parasites are all provided for by adults (e.g., social, progressive-provisioning (e.g., honey bees) or solitary, mass-provisioning (e.g., mason bees) Hymenoptera). As these immature insects generally don’t need to seek out resources or engage in defense behaviors prior to metamorphosis, they may not need the same capabilities that the adults that provision for/defend them require. Sóvik et al. (2015) suggest that differences in the degree of larval independence in two model insect systems (honey bees, and the fruit/vinegar fly, Drosophila melanogaster) may explain potential differences in their ability to associate rewards with stimuli. Larval D. melanogaster must seek out and assess food resources (Schwarz et al. 2014) and rely on their nociceptive neurons to engage in defense against parasitoids (Robertson et al. 2013); this degree of fitness-relevant, independent environmental interaction may explain why evidence of their ability to associate rewards and stimuli is abundant while evidence on reward pathways in the more dependent larval honey bees is currently non-existent (Sóvik et al. 2015).
Similarly, the capacity for sentience of commercially reared and wild insects may develop differently if there are key differences between these environments (even for individuals within the same lifestage). For example, an increasingly common management practice for honey bees is to move colonies across the country to pollinate different agricultural sites; this involves sealing the hive for the duration of transport which may delay the onset of foraging behaviors for younger bees. Adult honey bee neurological development is conditional on foraging experience - age-matched bees that were able to forage had greater mushroom body volumes than those that remained in the nest (Maleszka et al. 2009). This demonstrates that, for individuals confined to the hive and missing stimuli at critical points in development, traits related to mushroom body development and coordination can be stunted (Ismail et al. 2006).
Less is known about how immature rearing conditions affects experience-dependent structural plasticity in immature insect brains, but changes in the immatures’ rearing environments definitely can cause changes in adult neural structures (probably mediated via currently undescribed changes to larval neural structures). As just one of many examples, larval heat stress reduced mushroom body development in adulthood in D. melanogaster flies (Wang et al. 2018). Black soldier fly larvae often experience very high temperatures as a result of metabolic heat production and crowding in commercial conditions (reviewed in Barrett et al. 2022a); if D. melanogaster serve as a reliable model for black soldier fly brain development, then we may well expect reduced adult mushroom body volumes in adult black soldier flies as a result of the challenge of managing thermal stress in commercial rearing conditions. Insofar as researchers can identify the factors necessary for cognitive development in each species–such as the environmental complexity, temperature fluctuation, photoperiod, or access to other individuals–it should be possible to make very rough and highly tentative predictions about the impacts that rearing environments have on the probability of sentience and welfare ranges.
Conclusion
There are many knowledge gaps that make it difficult to assess the probability of sentience and welfare ranges—not just in insects, but in animals generally. Still, the evidence we’ve reviewed suggests that there are likely to be some differences across insect life stages, though given neuroanatomical similarities and the scores on the trait survey, there doesn’t seem to be an empirical basis for supposing that such differences are large. More research would need to be done to determine if the knowledge gaps can be filled by experimentation and examination of the entire life cycles of the species of interest. With that research in hand, we would be better positioned to assess whether the sentience- and welfare-range-relevant traits that we identified are actually absent or simply unexplored in immature life stages or whether they simply don’t develop until adulthood.
The research that would be required to fill in the gaps in our knowledge would need to focus primarily on improving our understanding of the functions of the traits across the entirety of the life cycle of the insect. Importantly, this means we need data from multiple time points across immature development. A limitation of the data currently available, and thus utilized in this report, is that often data are collected only at a single time point during the immature life stage (if collected at all). Often the last stage of immature development is chosen, as most techniques are easier to perform on these larger individuals; but this is not always the case (meaning that data in our review are not standardized in this manner), and this provides us with no evidence of how the probability of sentience might change across the larval stage.
Data on insect neuroanatomy do not support the idea that the nervous system is identical (in form or function) across the entirety of immature development in either holometabolous or hemimetabolous insects; there are changes in volume, cell numbers and connections, and more between the first and last immature stages (e.g., honey bees, Farris et al. 1999; black soldier flies, Barrett et al. 2022b; [hemimetabolous] cockroaches, Neder 1959). We did not analyze differences in the probability of sentience or welfare ranges across immature development; however, if mushroom bodies are sentience-relevant neural structures, it seems probable that early and late-stage immature insects could differ. For example, data from D. melanogaster suggest that the small number of mushroom body neuroblasts a fly has following embryogenesis generate many of the progeny Kenyon body cells that make up the mushroom bodies throughout the immature development period (including pupation), suggesting L1 and L3 (first and last larval instars) D. melanogaster could differ in their sentience-relevant brain cell populations (Urbach and Technau 2003, Ito & Hotta 1992). Data in honey bees also shows substantial mushroom body cell population developments in the last larval stages (Farris et al. 1999). So, it is possible there is relevant variation in brain cell populations across immature development that should be further researched.
Additional empirical research projects could further aid in understanding the probability of sentience and welfare ranges across the life cycle - for example, to establish additional evidence from behavioral, neuroanatomical, and gene expression patterns in both wild and reared populations of the insects of interest. Commercial rearing setups may have compounding effects on trait development over the life cycle due to environmental and other resource factors, requiring that additional care be put into assessing traits that have been observed in a controlled and standardized manner between stages and species. Additional consideration of the welfare ranges in a social superorganism, like a honey bee colony, may be necessary to entirely disentangle which traits are truly required at the individual level. The division of labor due to the caste system has large implications on the requirements for a particular individual throughout their development, as some traits are “outsourced” to another individual for the sake of the overall functioning of the colony.
In order to better assess the differences between life stages across insect species, research programs could examine changes in the brain, including neuron distribution, synaptic density or cell connectivity, relative brain region sizes, genetic expression profiles, cellular metabolic rate changes, and even the development of peripheral sensory structures that could influence welfare ranges. Combining 3D microscopy techniques with neurophysiological testing (such as microelectrode placement) at each life stage, these data can map the regions of the neural anatomy of the insects of interest to enable a comparative assessment between species. Investigation of gene expression patterns at these stages can provide information on the biomarkers regulating certain developmental processes. Employing high speed cameras to document the biomechanics, movements, and behaviors of the insects can enable additional gaps to be filled regarding avoidance and seeking behaviors, among others. In order to be truly comparative and realistic, it would be important to employ a combination of these techniques (and others) at standardized points in individual development. Doing so would bring us steps closer to understanding welfare-relevant traits across both life stages and species.
Acknowledgements
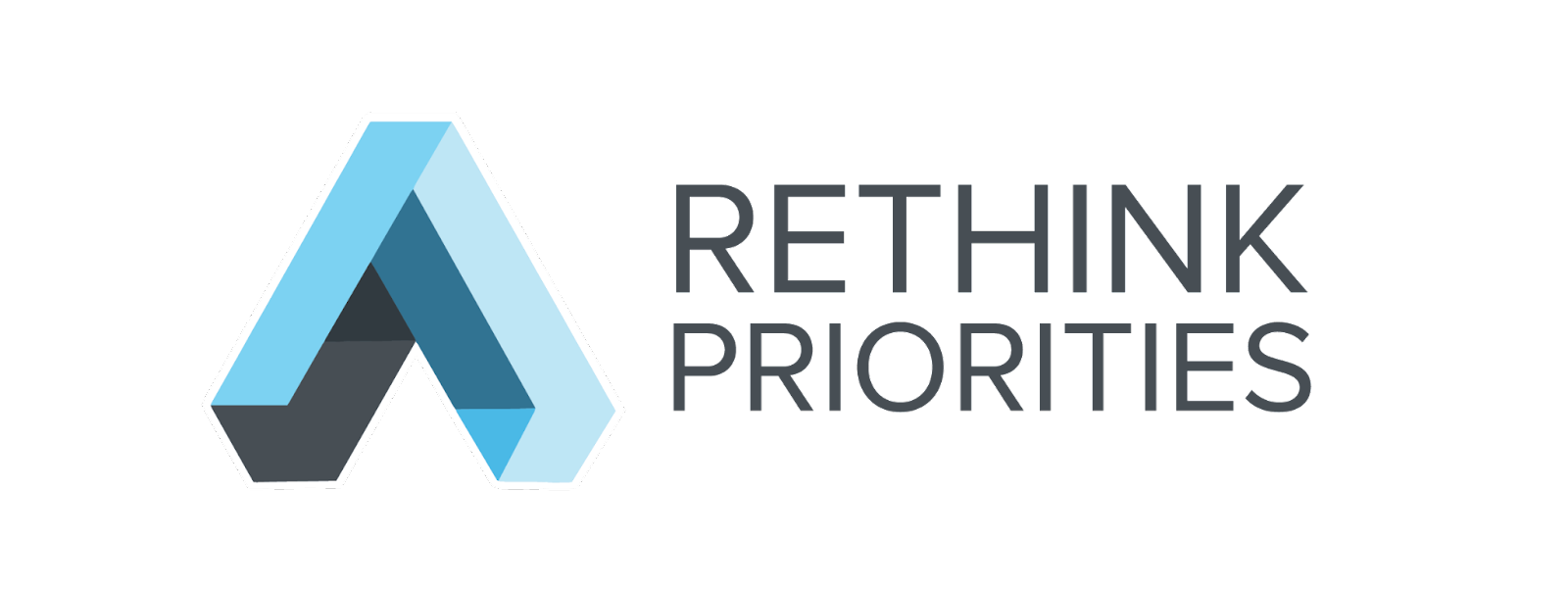
This research is a project of Rethink Priorities. It was written by Bob Fischer and Emily Sandall. For help at many different stages of this project, thanks to Meghan Barrett, Marcus Davis, Catarina Kissinger, and Jason Schukraft. If you’re interested in RP’s work, you can learn more by visiting our research database. For regular updates, please consider subscribing to our newsletter.
References
- Barrett M, Chia SY, Fischer B, Tomberlin JK (2022a) Farmed black soldier fly, Hermetia illucens (Diptera: Stratiomyidae) welfare considerations: A model for the insects as food and feed industry. https://osf.io/yd5bt/
- Barrett M, Godfrey RK, Sterner EJ, Waddell EA (2022b) Impacts of development and adult sex on brain cell numbers in the Black Soldier Fly, Hermetia illucens L. (Diptera: Stratiomyidae). https://www.biorxiv.org/content/10.1101/2022.01.25.477588v1.full
- Barron AB, Klein C (2016) What insects can tell us about the origins of consciousness. PNAS 113: 4900-4908.
- Barron AB, Corbet SA (1999) Preimaginal condition in Drosophila revisited. Anim Behav 58: 621-628.
- Blackiston DJ, Silva Casey E, Weiss MR (2008) Retention of memory through metamorphosis: Can a moth remember what it learned as a caterpillar? PLoS ONE 3(3): e1736.
- Cabirol A, Cope AJ, Barron AB, Devaud J (2018) Relationship between brain plasticity, learning and foraging performance in honey bees. PLOS One, 13: e0196749.
- Cayre M, Strambi C, Strambi A (1994) Neurogenesis in an adult insect brain and its hormonal control. Nature 368:57–59.
- Chittka L, Niven J (2009) Are bigger brains better? Curr Biol 19: PR995-R1008.
- Farris SM, Robinson GE, Davis RL, Fahrbach SE (1999) Larval and pupal development of the mushroom bodies in the honey bee, Apis mellifera. J Comp Neuro 414: 97-113.
- Farris SM (2008) Structural, functional and developmental convergence of the insect mushroom bodies with higher brain centers of vertebrates. Brain Behav Evol 72: 1-15.
- Farris SM, Strausfeld NJ (2001) Development of laminar organization in the mushroom bodies of the cockroach: Kenyon cell proliferation, outgrowth, and maturation. J. Comp. Neurol. 439:331–51.
- Fahrbach SE (2006) Structure of the mushroom bodies of the insect brain. Annual Review of Entomology 51: 209-232.
- Fernandez-Cassi X, Supeanu A, Vaga M, Jansson A, Boqvist S, Vagsholm I (2019) The house cricket (Acheta domesticus) as a novel food: a risk profile. Journal of Insects as Food and Feed: 5(2): 137-157
- Gandolfi M, Mattiacci L, Dorn S (2003) Preimaginal learning determines adult response to chemical stimuli in a parasitic wasp. Proc B 270: 2623-2629.
- Ganguly A, Qi C, Bajaj J, Lee D (2020) Serotonin receptor 5-HT7 in Drosophila mushroom body neurons mediates larval appetitive olfactory learning. Scientific Reports 10: 21267.
- Godfrey RK, Swartzlander M, Gronenberg W (2021) Allometric analysis of brain cell number in Hymenoptera suggests ant brains diverge from general trends. Proc. R. Soc. B 288: 20210199.
- Guo Y, Wang Y, Wang Q, Wang Z (2014) The Role of PPK26 in Drosophila Larval Mechanical Nociception. Cell Reports. 9(4): 1183-1190.
- Hedrick AV, Kortet R (2012) Sex differences in the repeatability of boldness over metamorphosis. Behav Ecol Sociobiol 66: 407-412.
- Hinton HE (1948) On the origin and function of the pupal stage. Transactions of the Royal Entomological Society of London, 99: 395-409.
- Howard SR, Greentree J, Avargues-Weber A, Garcia JE, Greentree AD, Dyer AG (2022) Numerosity categorization by parity in an insect and simple neural network. Front Ecol Evol, doi:10.3389/fevo.2022.805385.
- Im SH, Galko MJ (2012) Pokes, sunburn, and hot sauce: Drosophila as an emerging model for the biology of nociception. Developmental Dynamics. 241: 16-26.
- Isingrini M, Lenoir A, Jaisson P (1985) Preimaginal learning as a basis of colony-brood recognition in the ant Cataglyphis cursor. PNAS 82: 8545-8547.
- Ismail N, Robinson GE, Fahrbach SE (2006) Stimulation of muscarinic receptors mimics experience-dependent plasticity in the honey bee brain. PNAS 103: 207-211.
- Ito K, Hotta Y (1992) Proliferation pattern of postembryonic neuroblasts in the brain of Drosophila melanogaster. Developmental Biology 149: 134-148.
- Kohno K, Sokabe T, Tominaga M, Kadowaki T (2010) Honey bee thermal/chemical sensor, AmHsTRPA, reveals neofunctionalization and loss of transient receptor potential channel genes. J Neuroscience 30: 12219-12229.
- Lievens S, Poma G, De Smet J, Van Campenhout L, Covaci A, Van Der Borght M (2019) Chemical safety of black soldier fly larvae (Hermetia illucens), knowledge gaps and recommendations for future research: a critical review. Journal of Insects as Food and Feed: 7(4): 383-396.
- Malaterre J, Strambi C, Chiang AS, Aouane A, Strambi A, Cayre M. 2002. Development of cricket mushroom bodies. J. Comp. Neurol. 452: 215–227.
- Maleszka J, Barron AB, Helliwell PG, Maleszka R (2009) Effect of age, behaviour and social environment on honey bee brain plasticity. JCPA 195: 733-740.
- Milinkeviciute G, Gentile C, Neely GG (2012) Drosophila as a tool for studying the conserved genetics of pain. Clinical Genetics. 82(4): 359-366.
- Morales-Ramos JA, Rojas MG, Shapiro-Ilan DI, Tedder WL (2010) Developmental plasticity in Tenebrio molitor (Coleoptera: Tenebrionidae): Analysis of instar variation in number and development time under different diets. J Entomological Science 45: 75-90.
- Muratore IB, Fandozzi EM, Traniella JFA (2022) Behavioral performance and division of labor influence brain mosaicism in the leafcutter ant Atta cephalotes. JCPA 208: 325-344.
- Murray DRP (1960) The stimulus to feeding in larvae of Tenebrio molitor L. J Insect Physiol 4: 80-91.
- Neder R (1959) Allometrisches Wachstum von Hirnteilen bei drei verschieden großen Schabenarten. Zool. Jb. Anat. 77: 411–464.
- Neely GG, Hess A, Costigan M, Keene AC, Goulas S, Langeslag M, Griffin RS, Belfer I, Dai F, Smith SB, Diatchenko L, Gupta V, Xia C, Amann S, Kreitz S, Heindl-Erdmann C, Wolz S, Ly CV, Arora S (2010) A Genome-wide Drosophila Screen for Heat Nociception Identifies α2δ3 as an Evolutionarily Conserved Pain Gene. Cell. 143(4): 628-638.
- O’Donnell S, Bulova S, Barrett M (2021) Experience-expectant brain plasticity corresponds to caste-specific abiotic challenges in dampwood termites (Zootermopsis angusticollis and Z. nevadensis). Science of Nature 108: 57.
- Patton RL (1978) Growth and development parameters for Acheta domesticus. Annals of the Entomological Society of America 71: 40-42.
- Polilov AA (2012) The smallest insects evolve anucleate neurons. Arthropod Structure and Development, 41: 29-34.
- Ray S (1999) Survival of olfactory memory through metamorphosis in the fly Musca domestica. Neuro Letters 259: 37-40.
- Reitdorf K, Steidle JLM (2002) Was Hopkins right? Influence of larval and early adult experience on the olfactory response in the granary weevil Sitophilus granarius (Coleoptera, Curculionidae). Physiol Entomol 27: 223-227.
- Robertson JL, Tsubouchi A, Tracey WD (2013) Larval defense against attack from parasitoid wasps requires nociceptive neurons. PLoS ONE 8(10): e78704.
- Rolff J, Johnston PR, Reynolds S (2019) Complete metamorphosis of insects. Phil Trans R Soc B. 374: 20190063.
- Schwarz S, Durisko Z, Dukas R (2014) Food selection in larval fruit flies: Dynamics and effects on larval development. Naturwissenschaften 101: 61-68.
- Sóvik E, Barron AB (2015) Insect reward systems: Comparing flies and bees. In: Advances in Insect Physiology. DOI: 10.1016/bs.aiip.2014.12.006
- Thum AS, Gerber B (2019) Connectomics and function of a memory network: The mushroom body of larval Drosophila. Curr Opinion in Neurobiology 54: 146-154.
- Tully T, Cambiazo V, Kruse L (1994) Memory through metamorphosis in normal and mutant Drosophila. J Neuro 14: 68-74.
- Urbach R, Technau GM (2003) Early steps in building the insect brain: Neuroblast formation and segmental patterning in the developing brain of different insect species. Arthropod Structure and Development, 32: 103-123.
- Verlinden H (2018) Dopamine signalling in locusts and other insects. Insect Biochemistry and Molecular Biology. 97: 40-52.
- Walters E, Illich P, Weeks J, Lewin M (2001) Defensive responses of larval Manduca sexta and their sensitization by noxious stimuli in the laboratory and field. J Exp Biol 204: 457-469.
- Wang X, Amei A, de Belle JS, Roberts SP (2018) Environmental effects on Drosophila brain development and learning. J Exp Biol 221: jeb169375.
- Zhao X, Coptis V, Farris S (2008) Metamorphosis and adult development of the mushroom bodies of the red flour beetle, Tribolium castaneum. Developmental Neurobiology 68: 1487-1502.
- Zhong L, Hwang RY, Tracey WD (2010) Pickpocket Is a DEG/ENaC Protein Required for Mechanical Nociception in Drosophila Larvae. Current Biology. 20(5): 429-434.
Thank you very much for this post and all the other essays in the Moral Weight Sequence! They were a pleasure to read and I expect that I will revisit some of them many times in the future.
Hi Bob, thanks for this post, really interesting stuff.
I'm a researcher at the Shrimp Welfare Project. Do you have a sense of whether any of the broad conclusions are likely to apply to shrimps as well, or do you think it would require an entirely new research project?
Thanks,
Lucas
Great question, Lucas. My hunch is that all the broad conclusions probably apply, though I'd want to think through the details more carefully before standing behind that claim. I suppose one thing that really affects my thinking is whether the organism has to navigate its environment in search of resources. My impression is that the youngest shrimp aren't doing this; they're just being carried along like plankton. So, that lowers my estimation of their capacities to something more like grubs than juvenile crickets. But of course I haven't investigated this at all, so please don't put too much weight on that hot take!
Happy to discuss if it would be helpful; feel free to DM me.
Thanks very much Bob, appreciate the hot take! I'll get in touch if we'd like any more detail :)
https://www.science.org/doi/10.1126/science.add9330?utm_campaign=SciMag&utm_source=Twitter&utm_medium=ownedSocial just came out!
Thanks for sharing!
I think it would be nice to know what is the moral weight of the mean terrestrial and marine arthropod. I suppose the mean arthropod is quite:
Both these factors push the moral weight of the mean arthropod down, and therefore the estimates I gave here could significantly overestimate the importance of the welfare of arthropods (and nematodes).